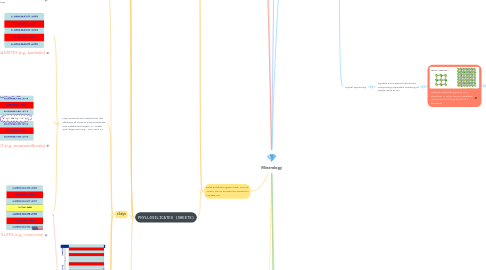
1. polymorphism
1.1. FRAMEWORK SILICATE (TECTOSILICATES)
1.1.1. feldspars (part 1)
1.1.1.1. low symmetry solids with either monoclinic or triclinic symmetry
1.1.1.2. metal site in pair in large holes in the structure, touching both sides of the hole
1.1.1.3. ALKALI FELDSPAR AT HIGH AND LOW TEMP
1.1.1.3.1. AT HIGH TEMP ALKALI FELDSPAR STABLE
1.1.1.3.2. AT LOW TEMP ALKALI FELDSPAR BREAKS DOWN (EXOLVES) INTO A MIXTURE OF TWO FELDSPARS (ALBITE AND ANRTHOCLASE). THIS INTERGROWTH = **PERTHITE**
1.1.1.4. K-FELDSPAR AT HIGH AND LOW TEMP
1.1.1.4.1. HIGH TEMP = **SANADINE**
1.1.1.4.2. ON COOLING FORMS A LOW TEMPERATURE FORM = **MICROCLINE (TARTAN TWINNING)**
1.1.2. feldspars (part 2)
1.1.2.1. comprise SiO4 tetrahedra joined to other tetrahedra at **every corner** creating an interconnected silicate framework
1.1.2.2. big ions (Na+, K+, Ca2+) in the gaps in the structure
1.1.2.3. frameworks are mixes of AlO4 and SiO4 tetrahedra
1.1.2.4. low symmetry- monoclinic or triclinic
1.1.2.5. general formula (Na,Ca,K)Al1-2Si3-2O8 end member formula (Al2Si3O8)
1.1.2.5.1. ANORTHITE CaAl2Si2O8
1.1.2.5.2. ALBITE NaAlSi3O8
1.1.2.5.3. ORTHOCLASE KAlSi3O8
1.1.3. Quartz (SiO2) and ice (H20)
1.1.3.1. both have structures based on tetrahedra joined at all four corners into 3D frameworks
1.2. phase diagrams
1.2.1. = graphical representations of the stability ranges of particular chemical compositions
1.2.2. show what we expect from theoretical studies = **thermodynamically stable state**
1.2.3. where the **stability fields** for each polymorph are expressed
1.3. polymorphism
1.3.1. the same chemical formula can be associated with more than one mineral different polymorphs **have the same chemistry but different structures**
1.3.1.1. e.g., graphite and diamond **C** quartz, tridymite, cristobalite, coesite, stishovite **SiO2** andalusite, sillimanite, kyanite **Al2SiO5**
1.3.2. high pressure minerals = denser than low pressure equivalents i.e., pressure squeezes the atoms closer together **Chatelier's principle** :arrow_backward:
1.3.2.1. :arrow_up: pressure = stabilise more dense structures
1.3.2.2. :arrow_up: temperature = stabilize less dense structure
1.3.2.2.1. **LE CHATELIER'S PRINCIPLE**
1.3.2.3. liquid structural states occur at higher temperatures
1.3.3. can be used as a **metamorphic indicator** can use the polymorph present in a rock to constrain the peak metamorphic pressure and temperature
1.3.3.1. SILLIMANITE forms in hot, low pressure regions such as next to hot igneous bodies
1.3.3.2. KYANITE forms at high pressure, high temperatures e.g., the Earth's lower crust
1.3.3.3. ANDALUSITE forms at moderate temperatures and pressures
1.3.4. SIO2 phase diagram **5 polymorphs**
1.3.4.1. tridymite and cristobalite = thermal metamorphism
1.3.4.2. coesite = deep mantle rocks
1.3.4.3. coesite and stishovite = meteorite impact craters (high pressure low temp)
1.3.4.4. covalently bonded
1.4. bonding in rocks
1.4.1. most rock forming minerals are covalently bonded. therefore most rocks are dominated by covalently bonded minerals. ionically bonded rocks = evaporates and limestone
1.4.2. covalently bonded minerals/rocks = strong = competent. deform in lower crust and mantle
1.4.3. ionically bonded minerals = weaker = less competent. deform in upper crust
1.4.3.1. if deform sedimentary sequence including sandstones (quarts, feldspar- covalently bonded) and limestone/evaporites (ionically bonded. the sandstones will remain competent but the evaporites will behave incompetently
1.4.3.1.1. e.g., during the alpine orogeny, 400-500m of Triassic evaporites were flattened into units a few m thick. the ionic rock acted as lubricant carrying relatively undeformed covalent rocks above it
1.4.4. hydrogen bonded minerals (ice) = bonds so weak that the material flows under its own weight - incompetent. deform anywhere on the earths surface. Allow ice to melt at ambient temperature and pressure. hydrogen bonds operate over long distances and therefore the resultant structure is very open
1.4.5. ICE
1.4.5.1. ice has a hexagonal symmetry and a structure very similar to quartz
1.4.5.2. where quartz is covalently bonded ice contains **hydrogen bonding** - polarity of H-O bond where O is slightly negative and H is slightly positive (weak bond hence ice is soft)
2. extra-terrestrial mineralogy
2.1. ICE
2.1.1. ice floats. in no other mineral system is the solid structural state LESS DENSE than the liquid. in all other natural mineral and rock systems, the solid would sink
2.1.1.1. **consequences of this behaviour** icebergs float pressure causes ice to melt ice can flow under its own weight- hydrogen bonds are insufficient to restrict mineral creep weak hydrogen bonds allow ice to melt at ambient temperature and pressure ice melts (and boils) at far lower temperatures than other minerals
2.1.2. ice properties cause the phase diagram to look different
2.1.3. profile through a glacier 1. the liquid phase more dense than solid 2. increase in pressure causes the more dense phase (water) to become stable
2.1.3.1. the thin film of liquid at the base of the glacier then causes the ice to flow more freely i.e., glaciers are self lubricating
2.2. bonding and geology (Venus vs earth vs titan vs pluto)
2.2.1. **TITAN**
2.2.1.1. gaseous, liquid and solid forms of methane exist on Titan **CH4**
2.2.1.1.1. At 100K and 0.5 atm all three phases of methane are possible
2.2.1.1.2. increases pressure causes methane to melt (like ice) and therefore methane glaciers may self lubricate
2.2.1.1.3. unlike water the methane bergs are in the bottoms of the ocean because the density of solid methane is greater than the density of liquid methane
2.2.1.1.4. van der waals bonding is the equivalent of ice i.e., CH4
2.2.1.2. rocks on Titan are made of **H2O**
2.2.2. VENUS
2.2.2.1. 100Bar and 750k
2.2.2.2. ionically bonded Galena condenses from the atmosphere on cooler, higher plateaus
2.2.2.3. ionic bonding is the equivalent of ice i.e., galena
2.2.3. **PLUTO**
2.2.3.1. Nitrogen works similarly to water pluto has nitrogen glaciers
2.2.3.2. ice is a rock and pluto has mountains made of ice
2.2.3.3. water (molten ice) acts like a magma and erupts in cryovolcanoes
2.2.3.4. surface phases on earth and titan are rocks on pluto
3. solid solutions gone mad- CLAYS AND MICAS EXOSOLUTIONS IN MINERALS
3.1. paired silicates (SOROSILICATE)
3.1.1. the most important sorosilicate mineral group is the **EPIDOTE GROUP** (low grade regional metamorphism)
3.1.1.1. contain the Si2O76- ions = DOUBLE SILICATE TETRAHEDRA and SiO4 double silicate tetrahedra
3.1.2. also includes zoisite (metamorphosed marls) and allanite (accessory in igneous rocks)
3.2. RING SILICATES (CYCLOSILICATES)
3.2.1. possible to get 3/5/6 and 9 tetrahedra ring
3.2.1.1. six fold rings = beryl (Cr bearing forms are emerald) and cordierite
3.3. PHYLLOSILICATES (SHEETS)
3.3.1. silicate tetrahedral share three corners to make a sheet
3.3.2. Al can swap for Si (aluminosilicate sheets)
3.3.3. sheets
3.3.4. and sheets are separated by IONS
3.3.5. **clays**
3.3.5.1. between **aluminosilicate** sheets are octahedral layers containing either Al or Mg
3.3.5.1.1. Mg = brucite layers = trioctahedral clays
3.3.5.1.2. Al = gibbsite layers = dioctahedral clays
3.3.5.2. clay minerals are made from the stacking of various aluminosilicate and octahedral layers +/- water and large ions e.g., Na+ and K+
3.3.5.2.1. KANDITES (e.g., kaolinite)
3.3.5.2.2. SMECTITES (e.g., montmorillonite)
3.3.5.2.3. ILLITES (e.g., muscovite)
3.3.5.3. in reality clays are interfingered sequences of all three end members = **mixed-layer clays**
3.3.5.4. Some ions transported in water are strongly attracted to the **surfaces** of clay minerals. =*adsorption* therefore accumulating pollutants and filters them from aquifers. however, is conditions such as pH change then the process can be *reversed. *
3.3.5.4.1. surfaces are highly charged and attract ions and metals to them
3.3.5.4.2. = adsorbed
3.3.5.4.3. clay nanocrystals are all surface and very little bulk therefore high adsorption.
3.3.5.5. morphology of clays in SEM
3.3.5.5.1. nanocrystals (less than 1micrometer) these are smaller than the wavelength of light so you cannot see them in thin section. they appear as turbidity or alteration in other minerals
3.3.5.5.2. clays are always nanocrystalline
3.3.6. micas (muscovite and biotite)
3.3.6.1. clays that grow crystals big enough to see i.e., clay with good crystallinity
3.3.6.2. micas = illite with a well formed and crystalline interlayer site
3.3.6.2.1. trioctahedral micas (with Mg=Fe2+) = BIOTITE
3.3.6.2.2. Dioctahedral micas (with Al and a vacancy) = MUSCOVITE
4. summary
4.1. isosilicates = olivine
4.2. chain silicate = pyroxene and amphibole
4.3. pair silicates = epidote
4.4. ring silicates = beryl and cordierite
4.5. layer silicates = clays, micas
4.6. framework silicates = feldspars
4.7. PROPERTIES OF MINERALS VS BONDING
4.8. bonding and geology
5. some common minerals systems
5.1. definitions
5.1.1. gem
5.1.1.1. highly prized (precious or semi-precious) earth material, usually (not always) a mineral. includes precious minerals (diamonds, rubies, sapphires- form of mineral corundum), precious metals (gold, silver..), semi-precious minerals (amethyst) and non-minerals (amber, jet, pearls)
5.1.1.2. what causes colour in gems?
5.1.1.2.1. 1. tiny amounts of **TRANSITION METALS** (e.g., Cr in Al2O3 corundum makes red ruby)
5.1.1.2.2. 2. tiny abouts of elements that create **HANGING BONDS** e.g. N5+ replacing C4+ in diamond makes it yellow)
5.1.1.2.3. 3. **RADIATION DAMAGE** e.g., smoky colour in smoky quartz
5.1.2. rock-forming minerals
5.1.2.1. silicates
5.1.2.1.1. vast majority of rock-forming minerals
5.1.2.1.2. SIO4 tetrahedron
5.1.2.1.3. silica (SIO2)
5.1.2.2. the most well known minerals in the earth (as opposed to gems or ore minerals)
5.1.3. solid solutions
5.1.3.1. when a mineral has a range of compositions between fixed limits. limiting compositions = end-members. compositions in between = solid solution
5.1.3.2. identified by putting brackets around the metals that can exchange
5.2. olivine (Mg, Fe)2SIO4
5.2.1. common mafic mineral
5.2.2. solid solutions between two endmembers FOSTERITE (Mg2SiO4) and FAYALITE (Fe2SiO4)
5.3. feldspars (Na,Ca,K)Al1-2Si3-2)8
5.3.1. most common mineral in the crust- 60% by volume
5.3.2. 3 end members, 2 solid solutions
5.3.2.1. the solid solution between albite and orthoclase = COMPLETE SOLID SOLUTION because all compositions between the end members are possible
5.3.2.2. the solid solution between Orthoclase and Anorthite has a large gap where compositions are not found = INCOMPLETE SOLID SOLUTION
5.3.2.3. OCCUR
5.3.3. often show twinning, exsolution and alteration in thin section
5.3.3.1. EXSOLUTION = Unmixing. Some homogeneous solid solutions of minerals are stable only at high temperatures. On cooling these become unstable and one mineral separates from the other at a certain temperature. An intergrowth of two separate minerals may result, e.g. PERTHITE, an intergrowth of sodium and potassium feldspar. This occurs without loss or addition to the mineral as a whole.
5.4. micas (K,Na)(Mg,Fe,Al)3AlSi3O10(OH)2
5.4.1. =SHEET SILICATE WHICH FORM PLATY HABITS
5.4.2. Mg, Fe rich micas = BIOTITE
5.4.3. Al rich = MUSCOVITE
5.5. Pyroxenes (Ca,Na,Mg,Fe,Al)2Si2O6
5.5.1. ORTHOPYROXENES
5.5.1.1. Mg, Fe rich (NO Ca)
5.5.1.2. orthorhombic symmetry
5.5.2. general
5.5.2.1. All 90o cleavage
5.5.2.2. more complex solid solutions with a general formula X2+Y2+(SiO6) where X and Y are Ca, Mg, Fe, Na, Al
5.5.2.3. note Ca2SiO6 (wollastonite) is NOT a pyroxene, but a PYROXENOID
5.5.3. CLINOPYROXENES
5.5.3.1. Ca, Fe, Mg Rich
5.5.3.2. monoclinic symmetry
5.5.4. classification diagram (pyroxene quadrilateral)
5.5.4.1. full diagram (Ca2,Mg2,Fe2)
5.5.4.2. diagram gets cut off
5.5.4.2.1. i.e.,
5.6. The 10 main rock forming minerals
5.6.1. in terms of structure these are all silicates and the SIO4 TETRAHEDRON CAN BE SHAPED INTO CHAINS, SHEETS OR FRAMEWORKS
5.6.1.1. I.E.,
5.6.1.2. I.E.,
6. nature of solid matter- minerals and rocks
6.1. definitions
6.1.1. crystalline
6.1.1.1. majority of natural materials- ordered in regular patten in 3D
6.1.1.2. most natural materials
6.1.1.3. e.g.,
6.1.1.4. e.g., atoms regularly ordered in three dimensions. long range order
6.1.1.5. why do crystalline solids form?
6.1.1.5.1. optimised bond distances
6.1.1.5.2. bond angles optimised
6.1.1.5.3. every atom in an optimum site
6.1.1.5.4. everything in its place
6.1.1.6. why do non crystalline solids form?
6.1.1.6.1. entropy requires some disorder in high temperature matter
6.1.1.6.2. if you freeze melts or solutions fast, they may not have time to crystallise, and form non-crystalline solids e.g., glass
6.1.1.6.3. sometimes crystalline solids can lose order if they contain radioactive elements effectively machine gunning the structure, smashing it up and making it amorphous
6.1.2. amorphous
6.1.2.1. totally disordered
6.1.2.2. atoms truly randomly ordered
6.1.3. glass (i.e., supercooled liquid)
6.1.3.1. some order inherited from the liquid state but not enough time to order into a crystal
6.1.3.2. e.g., atoms roughly the same distance apart and some areas with regular order. short range order (local bits where local coordination is not random)
6.1.3.3. GLASS=ROCK
6.1.4. mineral
6.1.4.1. naturally occurring solid material, usually crystalline (possibly amorphous) with a chemical composition which is essentially constant (within definable limits)
6.1.4.2. e.g., quartz, feldspar, mica
6.1.5. rock
6.1.5.1. naturally occurring aggregate of mineral grains which may include several different minerals
6.1.5.2. GLASS=ROCK (composition is very variable)
6.1.5.3. e.g., granite comprising of minerals quarts, feldspar, mica
6.2. crystal symmetry
6.2.1. crystals are ordered structures comprising repeated stacking of single units in 3D
6.2.1.1. individual building block of a structure = UNIT CELL= smallest indivisible building block of a structure
6.2.1.1.1. unit cells are divided into 7 CRYSTAL SYSTEMS
7. packing of ions
7.1. Bonding?
7.1.1. = the manner in which atoms/ions join together. this dictates PHYSICAL PROPERTIES
7.2. TYPES OF BONDING
7.2.1. 1. IONIC BONDING
7.2.1.1. electrostatic attraction of oppositely charged ions
7.2.1.2. strong: ELECTRON TRANSFER
7.2.1.3. positive ions = cations, negative ions = anions
7.2.1.4. formations of an ion from an atom is associated with a certain energy, which has to be put into the system for ionisation to happen
7.2.1.4.1. electronegativity and ionisation potential
7.2.1.5. ionic bonds form between elements with MAXIMUM DIFFERENCE IN IONISATION POTENTIAL OR ELECTRONEGATIVITY
7.2.1.5.1. E.G.,
7.2.1.5.2. e.g.,
7.2.1.6. Ions can be regarded as spheres, and crystal structures as packing of those spheres. since a sphere has a radius, we can define the term IONIC RADIUS to represent the size of the ion
7.2.1.6.1. interionic distances can be calculated- this only works if bonding is 100% ionic
7.2.1.6.2. interionic distance calculations are a poor assumptions for elements not bonded ionically i.e., SiO4 tetrahedron where the bonding is 50/50 ionic covalent
7.2.1.7. properties of ionic minerals
7.2.1.7.1. can sometimes be soluble in water
7.2.1.7.2. moderate hardness 3-5 on mohs scale
7.2.1.7.3. MULTIPLE PERFECT CLEAVAGES
7.2.1.7.4. high symmetry (cubic, trigonal)
7.2.2. 2. METALLIC BONDING
7.2.2.1. a sea of electrons surrounding ions
7.2.2.2. moderately strong: ELECTRON MOBILITY
7.2.2.3. e.g., native metals (Cu, Ag, Au)
7.2.2.4. properties of metallic metals
7.2.2.4.1. electrical conductivity (caused by electron mobility)
7.2.2.4.2. high symmetry (cubic or hexagonal) - essentially packing of ions
7.2.2.4.3. **malleability**- ions slip over each other and the electrons move to compensate charge
7.2.3. 3. COVALENT BONDING
7.2.3.1. overlap of electron orbitals
7.2.3.2. strongest: ELECTRON SHARING
7.2.3.3. e.g., diamond, rutile
7.2.3.4. pure covalent bonds only occur between elements of the SAME ELECTRONEGETIVITY OR IONISATION POTENTIAL I.E., Cl2. some bonds are mostly covalent e.g., HCl with some polarisation. covalent bonds are highly directional, and therefore, it is not possible to talk about spheres in the same way as with ionic bonds.
7.2.3.5. properties of covalent minerals
7.2.3.5.1. Strongest in nature
7.2.3.5.2. very high hardness
7.2.3.5.3. most rock-forming minerals are silicates dominated by hybrid covalent-ionic bonds. ionic minerals hardness 3-4 and covalent 8-10 therefore silicates have a hardness between both
7.2.3.5.4. low symmetry
7.2.3.5.5. HIGH REFRACTIVE INDEX (RI). this is due to how light passes through shared electrons
7.2.4. 4. VAN DER WAALS BONDING
7.2.4.1. transient fluctuations in electron density
7.2.4.2. weak: TRANSIENT ELECTRON FLUCTUATIONS
7.2.4.3. e.g., graphite- the carbon layers are held together by weak Van Der Waals forces, and therefore easily slip over each other
7.2.4.3.1. this explains the EXCELLENT CLEAVAGE and GREASY FEEL
7.3. The reality of bonding
7.3.1. most bonds are hybrids between two or more types e.g., 80% ionic 20% covalent. therefore some minerals will appear to show mixtures of properties e.g., pyroxenes hardness from the covalently bonded parts of the structure and excellent cleavage from the ionically bonded parts
7.3.2. general rules
7.4. making structures
7.4.1. 1. ALL ATOMS SAME SIZE (metals- close packing of spheres)
7.4.1.1. The driving force is density- i.e., get the most atoms as close together as possible
7.4.1.1.1. different BRAVAIS LATTICES have different densities i.e., the F-lattice is the most dense
7.4.1.1.2. metals have **dense structures** (usually close packed but occasionally body centred) this minimises bond distances and creates solids with the greatest density
7.4.1.2. STRUCTURE OF METALS (spheres of the same size)- close packed structures made of planes of spheres
7.4.1.2.1. 1. FACE CENTERED CUBIC (FCC) *denser *
7.4.1.2.2. 2. BODY CENTRED CUBIC (BCC) *less favoured *
7.4.2. 2. PACKING ATOMS OF DIFFERENT SIZES (minerals- ionic systems as spheres)
7.4.2.1. most minerals have more than one atom (ion) type and the ions will have different sizes. if the mineral is ionic we can consider its structure as the packing of spheres of different sizes
7.4.2.1.1. e.g., packing big ions and small ones
8. solid solutions
8.1. polymorphism
8.1.1. many polymorphic phases have a **cubic** form and a **hexagonal** form as they are based on FCC and HCP close packing of one ions with the other ions in the tetrahedral or octahedral site with the site being fully or partially occupied
8.1.2. in ZnS (perfectly ionic)
8.1.2.1. Wurtzite (hexagonal)
8.1.2.1.1. S2- ions in hexagonal close packing Zn2+ ions occupy half the tetrahedral voids
8.1.2.2. Sphalerite (Cubic)
8.1.2.2.1. S2- ions in cubic close packing Zn2+ occupy half the tetrahedral voids
8.2. where do ions fit and why?
8.2.1. small ions= tetrahedral
8.2.2. medium sized ions = either
8.2.3. large ions = octahedral
8.3. distorted structures
8.3.1. arise when there is a degree of covalency to the bonds i.e., the spheres do not pack perfectly
8.3.2. e.g., polymorphs of TiO2
8.3.2.1. anatase- tetragonal, FCC oxygen with tetrahedral sites filled
8.3.2.2. rutile- tetragonal, HCP oxygen with tetrahedral sites filled
8.3.2.2.1. note that these are not cubic and hexagonal, this is because the bonding is significantly covalent and so the structure distorts reducing symmetry
8.3.2.3. brookite- orthorhombic, FCC with half octahedral sites filled
8.3.3. covalent bonding in solids
8.3.3.1. unlike ionic solids, covalent bonds overlap in space. the shape of the orbitals is now the key factor in determining the bond angles
8.3.3.2. e.g., silica tetrahedra
8.3.3.2.1. symmetry is lowered is change the identity of one of the apices
8.3.3.2.2. **very important point**- adding covalent character to solids where there are lots of substitutions (like minerals) means that the bond angles move slightly away from 109.5o due to changes in electron densities and THEREFORE THE SYMMETRY IS REDUCED
8.3.3.2.3. The SiO4 tetrahedral becomes distorted readily if... **and therefore symmetry lowered**
8.3.3.3. note that covalently bonded solids are not always low symmetry i.e., diamond is covalent and cubic!
8.3.4. substitution of elements in structures
8.3.4.1. few minerals have pure chemical compositions, but vary depending on what elements happen to be around
8.3.4.2. however **structures exert controls** on which atoms are accepted into which minerals
8.3.4.2.1. GOLDSCHMIDT'S RULES
8.4. olivine
8.4.1. isosilicate (olivine)
8.4.1.1. SiO4 is a single tetrahedron separated by metals (Fe, Mg)
8.4.2. **=IDEAL SOLID SOLUTION**
8.4.2.1. two or more ions exchanging for each other without distortion of the structure
8.4.2.2. the properties of the members of an ideal solid solution can be calculated from a **linear combination of the properties of the end members= Vegard's law**
9. complex solid solutions
9.1. solid solutions with **3 end members** bold
9.1.1. Mn2+ has an ionic radius similar to Mg2+ therefore **there is a solid solution to Mn Olivine** Mn2SiO4 olivine (tephroite) can be synthesised
9.1.2. i.e.,
9.2. limited solid solution
9.2.1. = solid solution can occur to a certain degree but not to completion
9.2.2. e.g., Ca2+ substitution in Mg2SiO4 (forsterite olivine) does occur but **does not** go all the way to Ca2SiO4 because the Ca2+ ion is too big
9.2.2.1. therefore get 3 component incomplete solid solution
9.3. **homovalent substitution** :couple:
9.3.1. = swapping an ions of a particular charge with another of **the same charge**
9.4. structures with two different sites :v:
9.4.1. e.g., pyroxene (Ca,Mg,Fe2+)2Si2O6. with sites XYSi2O6
9.4.1.1. Site 1 = x = small (no Ca :x:) accommodates Mg and Fe2+
9.4.1.1.1. **ORTHOPYROXENES**
9.4.1.2. site 2 = y = bigger (Ca :ballot_box_with_check:) accommodates Ca, Mg and Fe
9.4.1.2.1. because Ca cannot fit in X, the Calcium end member must be CaMgSi2O6 i.e., Ca can occupy a maximum of one site. Ca2Si2O6 (wollastonite) does exist but has a different structure= **pyroxenoid**
9.4.1.2.2. **CLINOPYROXENES**
9.4.1.3. exchange mechanisms in pyroxene
9.4.1.3.1. **homovalent exchange ** Mn2+=Mg2+=Fe2+=Ca2+
9.4.1.3.2. Aliovalent substitution
9.5. Aliovalent substitution
9.5.1. you can exchange ions of different charges is the increase in charge in one place is countered by a decrease elsewhere **i.e., coupled substitution**
9.6. Chain silicates
9.6.1. Pyroxene
9.6.1.1. characterised by two prominent cleavages on the basal sections. Y sites are the weakest and therefore the structure breaks readily along them 90o to each other.
9.6.1.2. SiO4 tetrahedra lie in chains with metals in between (Mg, Fe, Ca). with chains stacking differently in clinopyroxene and orthopyroxene
9.6.2. Amphibole
9.6.2.1. double chains
9.6.2.2. **3** different sites (AXY)
9.6.2.2.1. site 1 = X = small (Mg, Fe, Mn)
9.6.2.2.2. site 2 = y = bigger (Ca)
9.6.2.2.3. Site 3 = A = huge (Na, K)
9.6.2.2.4. because tetrahedral Al exchanges for Si on the chains. this means
9.6.2.2.5. 5 different sites between the chains and the ability to swap tetrahedral Al for Si on the chain
9.6.2.3. cleavage (plane of weakness) is now at 120/60
9.6.2.4. Exchange mechanisms in Amphibole
9.6.2.4.1. **Homovalent exchange** Mn2+=Mg2+=Fe2+=Ca2+
9.6.2.4.2. **Aliovalent exchange** (same as for pyroxene)
9.6.2.5. AMPHIBOLE QUADRILATERAL
9.6.2.5.1. Monoclinic and orthorhombic amphibole
9.6.2.5.2. note there is no equivalent to pigeonite
9.6.2.6. amphibole vs pyroxene
9.6.2.6.1. similarities to pyroxene
9.6.2.6.2. unlike pyroxene
9.6.2.7. what is hornblende?
9.6.2.7.1. = amphibole equivalent of augite (Ca, Mg, Fe) + A site Na and therefore adding tetrahedral Al
9.6.2.8. **amphibole discrimination diagrams** created by comparing the occupancies of particular sites and create fields which explain the compositional variability within sites
9.6.2.8.1. as add more Al the Si content drops and A site goes from 0 to 1
9.6.2.8.2. sodic amphibole